This article describes the role of electrochemical gradients in photosynthesis, including electron transport and carbon fixation, as well as the impact of light quality on electron transport and ATP generation.
Electrochemical Gradients, Plant Metabolism, and Light Quality
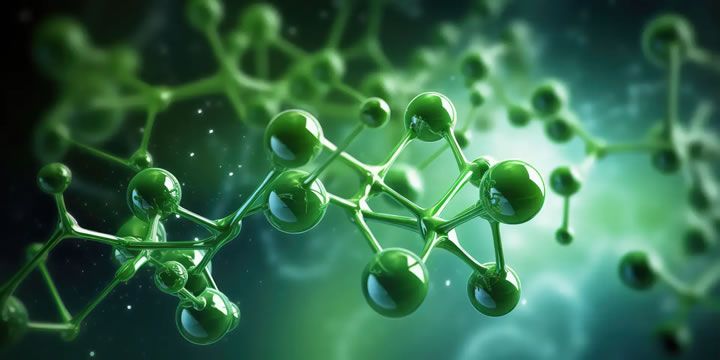
Jeff Bates | UbiGro
In the realm of greenhouse cultivation, understanding the intricate processes governing plant metabolism, including the creation of electrochemical gradients and ATP generation, is not just a matter of scientific curiosity; it is a pivotal factor that can significantly impact crop yield and quality. If you ask a grower to describe photosynthesis, they might tell you it’s how plants use sunlight to grow. But how the sunlight energy is transformed into chemical energy through the establishment of electrochemical gradients, ATP generation, and plant metabolism is a mechanism that fewer can explain. This article describes the role of electrochemical gradients in photosynthesis, including electron transport and carbon fixation, as well as the impact of light quality on electron transport and ATP generation.
Beyond the familiar concept of photosynthesis lies a complex interplay of electrochemical gradients (EG), specifically proton motive force, and ATP generation that orchestrates crucial biochemical reactions within plant cells, thus highlighting the vital connection between light quality, electrochemical gradients, plant metabolism, and ATP generation.
This article delves into the fascinating world of electrochemical gradients, unraveling their role in photosynthetic electron transport chains (PETC), the generation of ATP through proton motive force, and their influence on the activation of enzymes crucial for carbon fixation. As greenhouse growers aim to optimize conditions for plant growth, a comprehensive understanding of these processes becomes paramount.
Furthermore, this article explores the correlation between light quality and electron transport rate,
shedding light on how manipulating the spectral composition of light can be a strategic tool for
enhancing photosynthetic efficiency. By examining the impact of light quality on electron transport chains, this discussion equips greenhouse cultivators with insights into maximizing crop productivity through informed adjustments in lighting strategies.
Figure 1. An electrochemical gradient generated by hydrogen cations.
Electrochemical Gradients
An electrochemical gradient (EG) occurs when a chemical species, usually an ion, exists on both sides of a membrane (a permeable boundary in an organism) in different concentrations and charges (Figure 1).
If possible, ions will move from an area of higher concentration to lower concentration through passive diffusion. They also carry an electric charge, so their movement across membranes creates a transmembrane electrical potential. This results in a gradient that is not just chemical, but electrochemical. Simply put, when an EG exists, the exterior and interior of a plant cell hold different electrical potentials, forming a transmembrane potential, and changing the energy required to move across the membrane.
Figure 2. Inside of chloroplast inner membrane is the stroma, which contains lumen inside of thylakoids.
Photosynthetic Electron Transport Chains
Chlorophyll absorbs energy from sunlight and is involved in forming energy storage molecules (ATP and NADPH) in the light reactions of photosynthesis. Chlorophyll is present in thylakoid membranes which are compartments inside chloroplasts (Figure 2). The EG that drives photosynthesis spans across these thylakoid membranes and is modulated by photosynthetic electron transport chains (PETCs).
Electron transport chains (ETCs) consist of membrane-embedded proteins that easily accept or donate electrons. In photosystem II, energy received as light is transferred to a water molecule: the water molecule splits, yielding hydrogen ions, molecular oxygen, and a free electron to travel on the PETCs. The electron transport rate on the PETC is affected by light quality, described in the final section of this article.
As the PETC moves electrons in a specific direction across the thylakoid membrane, the electrical charges of the thylakoid interior (lumen) and exterior (stroma) shift. To balance this shift, protons (in the form of hydrogen ions) are pumped from the stroma into the lumen by the PETC molecules. In this manner, the PETC generates and maintains an electrically-charged gradient across the thylakoid membrane, balanced by hydrogen protons and free electrons (Figure 3).
Figure 3. Schematic diagram of electron/proton movement by ETC.
Proton Motive Force
When a plant receives light, electron/proton movement by the PETC results in a greater proton concentration in the lumen than in the stroma, generating an EG. Proton motive force (PMF) is another name for EGs of protons. This PMF allows for another protein embedded in the thylakoid membrane, adenosine triphosphate synthase (ATP, ATPase), to produce chemical energy in the form of ATP. As the ETC shuttles more hydrogen protons into the lumen, the protons naturally want to diffuse back towards their lower concentration.
As they do, their movement physically rotates ATPase, converting potential energy into kinetic energy, and combining adenosine diphosphate with inorganic phosphorus, yielding ATP (Figure 4). ATP is used for immediate energy needs or is turned into glucose for longer term storage.
Figure 4. ATPase exploits the transmembrane movement of protons to produce ATP.
This proton movement not only facilitates ATP production, but also changes pH. When the plant receives light, the PETC moves protons into the lumen, making the lumen environment more acidic. To mitigate the electric potential across the thylakoid membrane, magnesium cations diffuse across the membrane from the lumen into the stroma.
This affects the activity of ribulose-1,5-bisphosphate carboxylase/oxygenase (RuBisCO), an enzyme in the stroma that is responsible for carbon fixation. RuBisCO exists in a deactivated and activated state; when activated, it acts as a catalyst. The increase in pH and magnesium, resulting from hydrogen efflux and magnesium influx, creates a more preferable environment for RuBisCO activation. In this manner, the PETC not only regulates ATP synthesis, but also plays a role in carbon fixation.
Respiratory ETCs
Photosynthesis is not the only metabolic process driven by proton motive force. Proton gradients are also found in mitochondria, which break down glucose in the process of respiration. In contrast with the PETC, the respiratory electron transport chain (RETC) and ATPase are embedded in the inner mitochondrial membrane.
The RETC does not receive free electrons from hydrolysis, but rather from nicotinamide/ flavin adenine dinucleotides (NADH/FADH2) (Figure 5). The RETC shuttles protons from the mitochondrial matrix into the intermembrane space to compensate for electron movement. The result is carbohydrates produced through photosynthesis can later be broken down for energy as needed by the plant.
Figure 5. Schematic diagram of mitochondrial respiratory ETC.
Light Quality and Electron Transport Rate
Light quality refers to spectral wavelength composition: for example, an LED fixture might emit a light quality of 80% red (600-700 nm), 20% blue (400-500 nm). Plants demonstrate growth responses to light qualities in the range of plant biologically active radiation (BAR, 280-750 nm). BAR encompasses radiation that is ultraviolet (UV, 280-400 nm), photosynthetically active (PAR, 400-700 nm), and far-red (FR, 700-750 nm). PAR contributes directly to photosynthesis and is divided into wavelength ranges corresponding to visible colors: blue, green, and red (400-500, 500-600, 600-700 nm, respectively).
Red light is immediately directed to chloroplasts for photosynthetic processing; meanwhile, higher-energy PAR wavelengths (i.e., blue) are processed by accessory pigments (including carotenoids) to lose some energy before that energy is transferred to chloroplasts. High QY of red light has led to the development of photoconversion technologies that convert higher-energy BAR to red wavelengths (i.e., UV and blue to red). As a result, plant growth rates and yields increase.
Shorter PAR wavelengths (400-670 nm) typically excite PSII, meanwhile FR light (700-800 nm) preferentially excites PSI. When functioning of both photosystems is balanced, ETR is optimized, leading to increased photochemical efficiency (Figure 6). In this manner, studying light quality effects on plant growth should not only consider individual wavelength intensities separately, but also ratios of wavelengths (e.g. R:FR).
Figure 6. Electrons move from PSII to PSI on the electron transport
Electron transport chains not only move electrons, but also hydrogen protons that establish an electrochemical gradient necessary for photosynthesis and respiration. The proton electrochemical gradient generates proton motive force and regulates pH, facilitating ATP generation and RuBisCO activation. Light quality impacts the rate of electron transport on ETCs by affecting PSII and PSI activity.
Technologies that manipulate light quality must therefore consider light wavelength ratios that equally excite both photosystems and increase net photosynthesis. By recognizing the significance of electrochemical gradients and their interaction with light quality and plant metabolism, greenhouse cultivators are empowered to maximize the productivity of their crops through thoughtful and targeted environmental management, focusing on ATP generation within the context of plant metabolism.
References
Duysens, L. N. M., & Amesz, J. (1962). Function and identification of two photochemical systems in photosynthesis. Biochimica et Biophysica Acta, 64(2), 243-260.
Evans, J. R. (1987). The dependence of quantum yield on wavelength and growth irradiance. Functional Plant Biology, 14(1), 69-79.
Evans, J. R., Morgan, P. B., & von Caemmerer, S. (2017). Light quality affects chloroplast electron transport rates estimated from Chl fluorescence measurements. Plant and Cell Physiology, 58(10), 1652-1660.
Hogewoning, S. W., Wientjes, E., Douwstra, P., Trouwborst, G., Van Ieperen, W., Croce, R., & Harbinson, J. (2012). Photosynthetic quantum yield dynamics: from photosystems to leaves. The plant cell, 24(5), 1921-1935.
McCree, K. J. (1971). The action spectrum, absorptance and quantum yield of photosynthesis in crop plants. Agricultural Meteorology, 9, 191-216.
Myers, J. (1971). Enhancement studies in photosynthesis. Annual Review of Plant Physiology, 22(1), 289-312.
Su, J. H., & Shen, Y. K. (2005). Influence of State-2 transition on the proton motive force across the thylakoid membrane in spinach chloroplasts. Photosynthesis research, 85, 235-245.
Zhen, S., & van Iersel, M. W. (2017). Far-red light is needed for efficient photochemistry and photosynthesis. Journal of plant physiology, 209, 115-122.
The content & opinions in this article are the author’s and do not necessarily represent the views of AgriTechTomorrow
Comments (0)
This post does not have any comments. Be the first to leave a comment below.
Featured Product
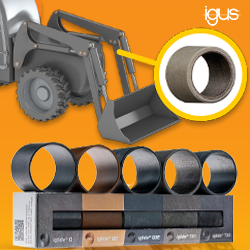